Team:Waterloo/Project
From 2011.igem.org
You can write a background of your team here. Give us a background of your team, the members, etc. Or tell us more about something of your choosing. | File:Waterloo logo.png 200px |
Tell us more about your project. Give us background. Use this is the abstract of your project. Be descriptive but concise (1-2 paragraphs) | File:Waterloo team.png Your team picture |
Team Example |
Home | Team | Official Team Profile | Project | Parts Submitted to the Registry | Modeling | Notebook | Safety | Attributions |
---|
Contents |
Project
Introduction
The goal of Waterloo's 2011 iGEM project is to implement self-excising ribozymes (introns) as biobricks. But first, what are self-excising ribozymes? Ribozymes are ribonucleic acid (RNA) enzymes and enzymes are reaction catalysts. So ribozymes are just RNA sequences that catalyze a (trans-esterification) reaction to remove itself from the rest of the RNA sequence. Essentially these are considered introns, which are intragenic regions spliced from mRNA to produce mature RNA with a continuous exon (coding region) sequence. Self-excising introns/ribozymes consist of type I and II introns. They are considered self-splicing because they do not require proteins to intitialize the reaction.
What are biobricks? Standard biological parts such as DNA sequences of known structure and function that can be incorporated into living cells to construct new biological systems. Therefore, by understanding the sequences and structure of these self-excising introns and making them useable, we can use them as tools to make other experiments easier.
A Little Bit About Group I Introns
All group I introns in bacteria have presently been shown to self-splice (with few exceptions) and maintain a conserved secondary structure comprised of a paired element which uses a guanosine (GMP, GDP or GTP) cofactor. Conversely, only a small portion of group II introns have been verified as ribozymes (they are not related to group I introns) and generally have too many regulators to easily work with. It is mainly the structural similarity of these introns that designates them to group II. We will mainly be working with group I introns, such as the phage twort.ORF143.
Group I introns contain a conserved core region consisting of two helical domains (P4–P6 and P3–P7). Recent studies have demonstrated that the elements required for catalysis are mostly in the P3 to P7 domain. They are ribozymes that consecutively catalyze two trans-esterification reactions that remove themselves from the precursor RNA and ligate the flanking exons. They consist of a universally conserved core region and subgroup-specific peripheral regions, which are not essential for catalysis but usually cause a reduction in catalytic efficiency if removed. To compensate for this, a high concentration of magnesium ions, spermidine or other chemicals that stabilize RNA structures can be added. Thus, the peripheral regions likely stabilize the structure of the conserved core region, which is essential for catalysis.
Trans-Esterification Reactions
The secondary structures, such as P6, formed by group I introns facilitates base pairing between the 5' end of the intron and the 3' end of the exon, as well as generates an internal guide sequence. Additionally, there is a pocket produced to encourage binding of the Guanosine cofactor. The Guanine nucleotide is placed on the first nucleotide of the intron. The 3'OH of Guanosine group nucleophilically attacks and cleaves the bond between the last nucleotide of the first exon and the first nucleotide of the 5' end of the intron; concurrently, trans-esterification occurs between the 3'OH and the 5'phosphorous from the 5' end of the intron. Subsequent conformational rearrangements ensure that the 3'OH of the first exon is placed in proximity of the 3' splice site. In this way, further trans-esterification reactions and splicing occurs.

Basic Biology Background
Some of the most innovative processes rely on the manipulation of routine processes. Biology is no exception. By understanding the basis of gene expression, we can manoeuvre a naturally occurring sequence (such as introns) to maximize our design needs. Protein can be thought of as the final product of a series of steps in a process called Gene Expression. Generally, genes are stored in the form of DNA, transcribed into RNA, the RNA is modified and often translated into protein. Our project allows us to manipulate this process to restrict sequences to particular levels of expression.
Transcription
Gene expression can be explained in a few different steps. First, it is pertinent to understand how genetic information is stored, which in many organisms is in the form of DNA (deoxyribonucleic acid). If DNA is a "language" then it uses these four letters (or bases): A (Adenine), T (Thymine), C (Cytosine) and G (Guanine). A always bonds with T and C always bonds with G.
If DNA is a "language" then RNA (ribonucleic acid) is a different dialect in which T is replaced with U (Uracil). The process of transcription converts DNA into RNA so that other processes can occur and this sequence can be read and understood by other molecules such as ribosomes. Transcription makes an RNA copy of all the DNA and this primary transcript is called pre-mRNA or pre-messenger RNA. Therefore, all the information strored in the DNA sequence is initially converted into the RNA sequence. However, certain sequences may be restricted to this level of expression and do not remain in the sequence for long. Sequences naturally restricted to this level are often referred to as introns. This is when post-transcriptional modification occurs.
Retrieved June 24, 2011 from Genetics Primer [2]
This diagram shows an overview of protein expression, where several proteins are coded for in this sequence.
Splicing
The pre-mRNA is edited before the actual protein is made in a process called splicing or post-transcriptional modification. This occurs when introns (regions of the DNA or RNA that do not code for the protein) are spliced out of the sequence (removed) and the exons (coding regions) are spliced (connected/ligated) together. Most of human DNA is made up of introns, however, this changes depending on the organism. Depending on the type of intron, some require the aid of other proteins to do so and others are self-splicing (Group I Introns). Self-excision occurs through a process called trans-esterification. This will result in mature mRNA. Our project utilizes this process to restrict sequences from moving beyond the RNA level, specifically by using the parts of self-excising introns necessary for excision. By this method, regions in between the necessary intron parts are removed from a sequence akin to the removal of an intron in the normal splicing process (see experimental design).
Retrieved June 24, 2011 from Genetics Primer [3]
This diagram shows the process of transcription, modification (splicing) and translation.
Restriction Enzymes
An essential tool to synthetic biology and biotechnology is the knowledge and implementation of restriction enzymes. Restriction endonucleases (or enzymes) cut double stranded DNA at a specific restriction site (a series of bases: ATCG etc...) and are typically named by the organism from which they were isolated: for example, EcoRI was isolated from E.Coli. Some restriction enzymes cut symmetrically through the double strand of DNA to produce a blunt-ended fragment (such as HindIII). Other enzymes cleave the DNA phosphodiester backbone at specific sites that produce sticky ends, which easily ligate to other frangments with compatible ends. EcoRI is an example of a restriction enzyme that produces a sticky-ended fragment. By knowing where specific enzymes cut, we can fragment DNA to have a specific sticky end or be of a specific length.
This is extremely useful in designing constructs so that they can contain whatever is necessary for the experiment. Complimentary ends provide an easy basis to ligate parts together. Restriction enzymes are used several times in this project, particularly with the construction of our experimental plasmid and experimental controls (see Project and Modeling). The experimental plasmid construction requires the use of SacI, EarI and SapI.
Retrieved July 4, 2011 from The Science Creative quarterly [4]
Translation
If DNA and RNA are different dialects of the same language then the expression of a protein is the translation of nucleic acid "language" into a protein "language". Although protein expression can be the final product of gene expression, many proteins undergo post-translational modification in which other components may be added or removed. Proteins are made up of amino acids that form a primary, secondary, tertiary or quaternary structure. Simply, translation is the process of assembling proteins based on the mRNA code by a ribosome. Other molecules are also involved in this process, such as transfer RNAs (tRNA), which bring the matching amino acids (as per the mRNA) to the ribosome to be assembled. Although protein expression is not always the product of gene expression, in our design it is the most easily quantified since a protein product provides an indication as to whether excision of the introns has occured properly. Green fluorescent protein (GFP) is a suitable choice for this design since fluorescence is easy to detect (under an ultraviolet (UV) light). Simply, if no fluorescence is visible then the experiment did not work. Nevertheless, ribozymes are an example of sequences that do not have a protein product. By definition these sequences are removed at the RNA level, are composed of catalytic RNA and are essentially restricted to this dialect.
Project Details
Experimental Design
Our protocol will involves the insertion of a functional protein, split by the self-removing elements, between CUCUUAGU and AAUAAGAG in the P6 region of twort.ORF143. GFP (green fluorescent protein) is split into two parts, which will be referred to as GFP1 and GFP2. With a constitutive promoter, GFP1 and GFP2 will be separated by a class 1 A2 intron split into two (for now, IN1 and IN2) sequences that flank another sequence inserted into the P6 loop, which was chosen because anything attached to this region will remain outside the protein. Note that this experimental design also contains an in frame stop codon, which is expected to be spliced out of the sequence with IN1 and IN2 and will utilize the RFC53 convention. Following GFP2 is a transcriptional terminator (TT). The method of making this construct is detailed in RFC53 and will be cut with EarI, SacI, SapI and Bg1II.
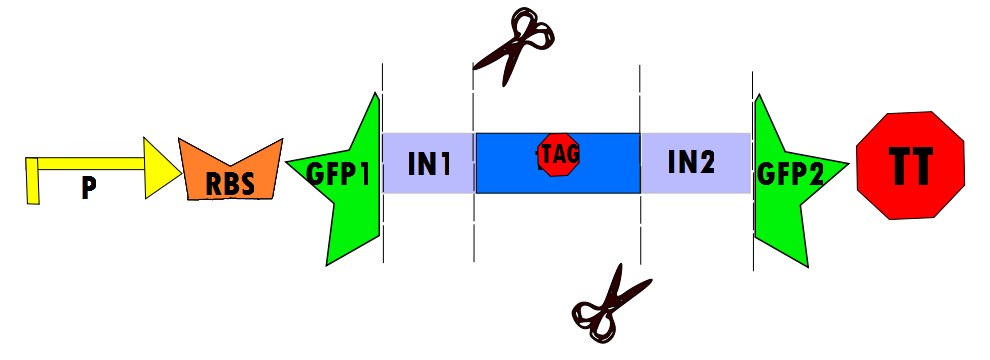

Making the Construct with RFC53
Figure 6 is a flow chart of the general work flow involved in the construction of our experimental plasmid, as per RFC53 conventions. The enzymes used are SacI, EarI and SapI, however, Bg1II could have been used if ligation were done in the opposite direction.
- (1) The insert is isolated through a series of enzyme digestions. As shown, the plasmid is initially cut by SacI. One Intron (in blue) is shown here as a representation. Note that SacI is the first enzyme to cut in the meta-prefix, instead of EarI because of the TCGA complementary overhand needed for ligation in (3). Next, EarI cuts at the meta-suffix to produce the CCA overhang also necessary for the step (3) ligation. The desired intert (containing the intron) is isolated for subsequent use.
=Mathematical Modeling= [under construction]
-free energy? -GFP output, GTP/Mg cofactor input?
- (2) Similarly, the PSB1C3 vector is isolated through enzyme digestion. Note that "N" indicates that this is the vector portion. SapI cuts in the meta-prefix region to produce the GGT overhang (complimentary to CCA in (1)). Next, SacI further cuts in the meta-prefix to produce the other sticky end (AGCT). The vector is also isolated for the ligation step.
- (3) The two components (insert and vector) are ligated together to produce the final construct.
- (4) According to the experimental design, the final construct will contain self-excising ribozymes, which in the last step result in a non-disruptive ligation scar and, therefore, the expression of GFP.
Sequences:
- Promoter-RBS-GFP1-metasuffix (588 nt)
GAATTCGCGG | CCGCTTCTAG | AGTTTACAGC | TAGCTCAGTC | CTAGGTATTA | TGCTAGCTAC |
TAGAGAAAGA | GGAGAAATAC | TAGATGCGTA | AAGGAGAAGA | ACTTTTCACT | GGAGTTGTCC |
CAATTCTTGT | TGAATTAGAT | GGTGATGTTA | ATGGGCACAA | ATTTTCTGTC | AGTGGAGAGG |
GTGAAGGTGA | TGCAACATAC | GGAAAACTTA | CCCTTAAATT | TATTTGCACT | ACTGGAAAAC |
TACCTGTTCC | ATGGCCAACA | CTTGTCACTA | CTTTCGGTTA | TGGTGTTCAA | TGCTTTGCGA |
GATACCCAGA | TCATATGAAA | CAGCATGACT | TTTTCAAGAG | TGCCATGCCC | GAAGGTTATG |
TACAGGAAAG | AACTATATTT | TTCAAAGATG | ACGGGAACTA | CAAGACACGT | GCTGAAGTCA |
AGTTTGAAGG | TGATACCCTT | GTTAATAGAA | TCGAGTTAAA | AGGTATTGAT | TTTAAAGAAG |
ATGGAAACAT | TCTTGGACAC | AAATTGGAAT | ACAACTATAA | CTCACACAAT | GTATACATCA |
TGGCAGACAA | ACAAGGTTGA | AGAGATCTAC | TAGTAGCGGC | CGCTGCAG |
- IN1 (172 nt)
GAATTCGCGG | CCGCTTCTAG | AGGAGCTCTT | CAGGTAAATA | ATTGCCTCTT | TATACAGTAA |
TGTATATCGA | AAAATCCTCT | AATTCAGGGA | ACACCTAAAC | AAACTAAGAT | GTAGGCAATC |
CTGAGCTAAG | CTCTTAGTAT | GTGAAGAGAT | CTACTAGTAG | CGGCCGCTGC | AG |
- IN2 (215 nt)
GAATTCGCGG | CCGCTTCTAG | AGGAGCTCTT | CAGGTAATAA | GAGAAAGTGC | AACGACTATT |
CCGATAGGAA | GTAGGGTCAA | GTGACTCGAA | ATGGGGATTA | CCCTTCTAGG | GTAGTGATAT |
AGTCTGATCA | TATATGGAAA | CATATAGAAG | GATAGGAGTA | ACGAACCTAT | TCGTAACATA |
AATGTGAAGA | GATCTACTAG | TAGCGGCCGC | TGCAG |
- metaprefix-GFP2-TT (442 nt)
GAATTCGCGG | CCGCTTCTAG | AGGAGCTCTT | CAATGAAGAA | TGGAATCAAA | GTTAACTTCA |
AAATTAGACA | CAACATTGAA | GATGGAAGCG | TTCAACTAGC | AGACCATTAT | CAACAAAATA |
CTCCAATTGG | CGATGGCCCT | GTCCTTTTAC | CAGACAACCA | TTACCTGTCC | ACACAATCTG |
CCCTTTCGAA | AGATCCCAAC | GAAAAGAGAG | ACCACATGGT | CCTTCTTGAG | TTTGTAACAG |
CTGCTGGGAT | TACACATGGC | ATGGATGAAC | TATACAAATA | ATAATACTAG | AGCCAGGCAT |
CAAATAAAAC | GAAAGGCTCA | GTCGAAAGAC | TGGGCCTTTC | GTTTTATCTG | TTGTTTGTCG |
GTGAACGCTC | TCTACTAGAG | TCACACTGGC | TCACCTTCGG | GTGGGCCTTT | CTGCGTTTAT |
ATACTAGTAG | CGGCCGCTGC | AG |
The Experiments
Positive Control
In the positive control, GFP1 and GFP2 flank either RFC25 or RFC53, which will not disrupt translation regardless of the linker. Therefore, fluorescence is expected. The experimental run will ideally show fluorescence resulting from the self-excision of IN1 and IN2.
Negative Control
In the negative control (using the same constitutive promoter), GFP1 and GFP2, followed by a transcriptional terminator, flank RFC10 (Request For Comments) resulting in a stop-codon-containing scar. No fluorescence is expected for this component (background) because translation is interrupted.
Biobricks Submitted
The following is a construction diagram showing the experimental design:
- K576005 contains the first component of GFP (GFP1)
- K576003 contains the first part of the intron sequence (IN1)
- J61046 contains the lox site
- K576006 contains the second component of GFP (GFP2)
- K576004 contains the second part of the intron sequence (IN2)
- K576011 contains the promoter (P), ribosomal binding site (RBS), GFP1, IN1, lox site, IN2, GFP2 and transcriptional terminator (TT). This is the final construct (experimental design).
This figure shows the construction of the positive and negative controls
- K576005 contains the first component of GFP (GFP1)
- K576006 contains the second component of GFP (GFP2)
- K576013 contains the promoter (P), ribosomal binding site (RBS), GFP and transcriptional terminator (TT). This is the positive control.
- K576005 contains the first component of GFP (GFP1)
- J61046 contains the lox site
- K576006 contains the second component of GFP (GFP2)
- K576012 contains the promoter (P), ribosomal binding site (RBS), GFP1, lox site, GFP2 and transcriptional terminator (TT). This is the negative control.